COSINE-100 Sets the Controls for the Heart of the Sun
By Shawn Westerdale, June 12, 2019
Article: “A search for solar axion induced signals with COSINE-100”
Authors: The COSINE-100 Collaboration
Reference: arXiv:1904.06860 [hep-ex]
I was torn between giving this post the current title and “Careful with the Axion, Eugene” — I’ve been on a bit of a Pink Floyd kick lately. Did I make the right choice in title? You decide.
Following my now-two-article-long trend of writing about WIMP direct detection experiments performing searches for physics beyond WIMPs, I wanted to write about some recent results from the COSINE-100 detector, located at Yangyang Underground Laboratory, in South Korea.
While COSINE-100’s main function is as a WIMP detector looking for an annularly modulating signal, their very low background rate and energy threshold has allowed them to perform a search for axions coming from the sun. So what are axions? And what is COSINE-100? And what did they find?
What are axions?
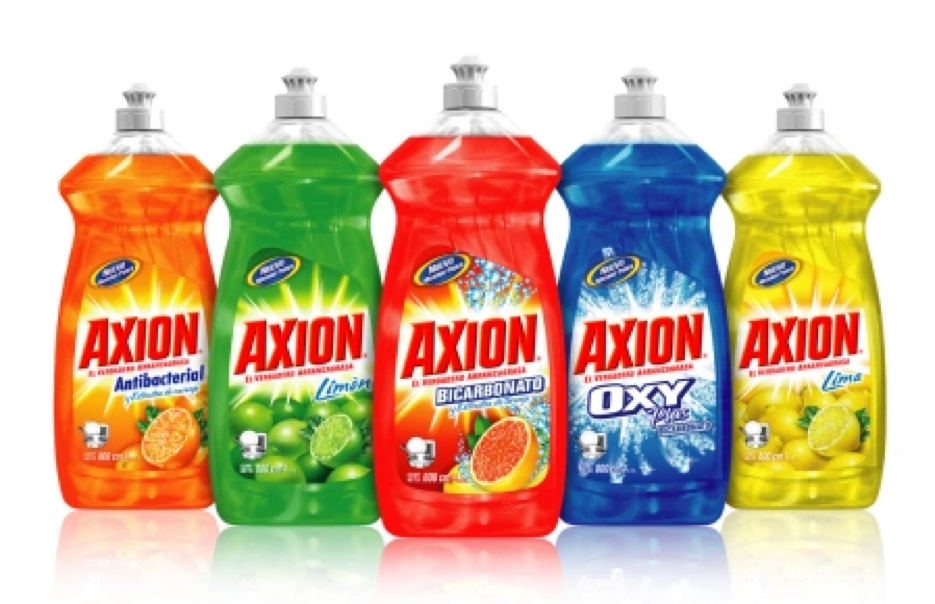
Figure 1. A photograph of axions observed at a local supermarket. Image source.
Axions are a type of particle originally motivated by the lack of CP violation observed in Quantum Chromodynamics (QCD). Interactions obeying CP symmetry means that if you flip the signs of the charge (C) of all particles in the interaction and you multiply all spatial coordinates by -1 (P, for “parity”), the equations describing the reaction should be unchanged. This would be like Alice walking through the looking glass and coming out as anti-Alice, just to find everything in Wonderland is exactly as it is in the normal world. The Standard Model says that QCD should include interaction terms that violate CP symmetry. One such interaction would result in the neutron having an electric dipole moment. While the neutron is electrically neutral, it is made of charged quarks that electrically balance each other out; the neutron’s spin coupled with these charges generates a magnetic dipole moment. If you play time in reverse, the direction of the spin changes, and so the magnetic dipole moment reverses (flipping under T symmetry); however, if you flip the spatial coordinates (transform under P), the magnetic dipole moment is unchanged. Therefore, the magnetic dipole moment reverses under PT transformations (it is “PT” odd). On the other hand, an electric dipole moment would be generated by a spatial separation of the internal charges. So if you apply a P transformation, the positive and negative charges swap and the electric dipole moment reverses. However, reversing time doesn’t change anything. As a result, the electric dipole moment is also PT odd, but for the opposite reason from the magnetic dipole. If you apply a CP transformation to the neutron (reverse the spatial coordinates and charges, but leave time unchanged), the magnetic dipole moment flips under C but not under P. The electric dipole moment flips under C and then flips back under P, and so ends up unchanged. Since the neutron’s behaviour in a strong electromagnetic field depends on both its dipole moments, the equations governing the neutron would change under these transformations. This means that the neutron as a whole does not obey CP symmetry if it has both electric and magnetic dipole moments. There are terms in QCD that should generate both of these, and a magnetic dipole moment is observed. However, very high precision measurements looking for the electric dipole moment, have found that it must be extraordinarily small, if it exists at all. It’s almost as if CP symmetry is forcing the electric dipole moment to zero — but why would that happen? In theory QCD should allow for CP violation! Since this conundrum affects the strong sector, it is generally referred to as the “strong CP problem”. In the 1970’s, Roberto Peccei and Helen Quinn found that this lack of CP violation could be explained by an additional chiral symmetry in the Standard Model.
A side effect of this symmetry breaking is that it would produce a bosonic field, with a very low mass. This corresponding particle is referred to as an axion, and it was later found that, depending on its mass and coupling constant, it could also be a candidate for cold dark matter.
The axion is predicted to have some rather peculiar properties that make it possible to design a detector to detect. In particular, it can couple to two photons, and in a strong electromagnetic field, it may turn into a photon and participate in electromagnetic interactions.
The idea of a very low mass particle such as this has been generalized to the axion-like particle, which is any light mass boson with properties like those described above. Not all of such particles can satisfy the conditions needed to be cold dark matter, and not all of them offer solutions to the strong CP problem. Those that do solve this problem are often called QCD axions, and a handful of QCD axion models directly relate the axion’s mass to its coupling constant to electrons and photons.
Additionally, Colgate found that axions can serve as a cleaning detergent, as illustrated above in Figure 1.
As you may have heard, the sun is very big. It also contains a very strong electromagnetic field, and it is a rich source of nuclear reactions. As such, a number of interactions between electrons, photons, and atoms in the sun can produce axions. So if you want to build a detector to search for axions, you can turn to the sun as a potentially very bright source. These solar axion searches are becoming a popular way of probing the parameter spaces in which axions and axion-like particles may live.
What is COSINE-100?
COSINE-100 is a detector made with a total of 106 kg of NaI crystals submerged in a liquid scintillator veto. This is all contained in lead and copper shielding and covered in a set of plastic scintillator panels that serve as a muon veto.
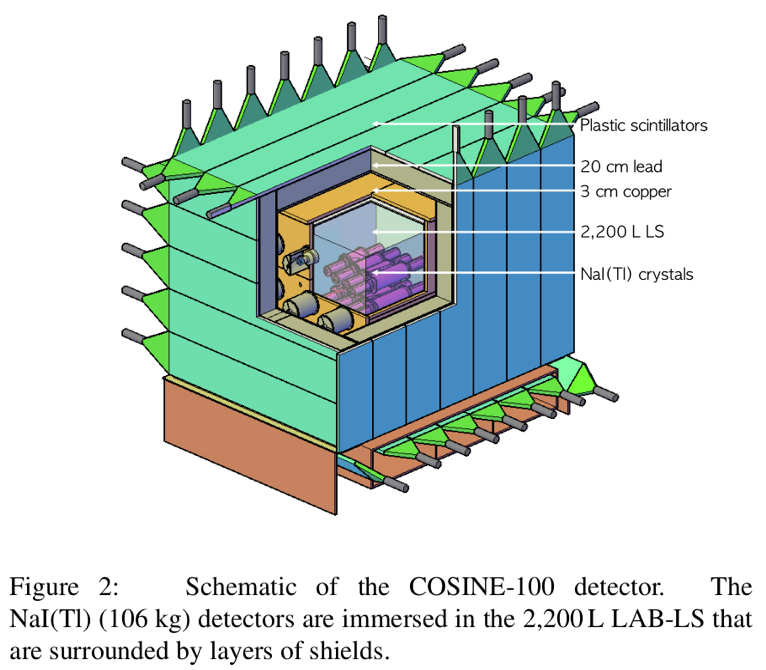
Figure 2 and caption from the paper.
The primary goal of the experiment is to look for WIMPs that scatter on Na or I nuclei in the detector and produce a scintillation signal. They do this by making extremely radio-pure NaI crystals with a low threshold. In their paper, they report a threshold of 2 keV for six of their eight crystals, and thresholds of 4 keV and 8 keV for the remaining two.
The use of NaI detectors was motivated by the DAMA-LIBRA experiment, operating at Laboratori Nazionali del Gran Sasso in Italy. This NaI detector has now been taking data for multiple decades, and they report to very high significance an observation of a low energy signal that modulates in rate with a frequency of one year. This modulation is consistent with what we’d expect from dark matter; as the earth travels around the sun its velocity relative to the dark matter varies over the course of the year. However, the interpretation of this signal as being from dark matter, rather than some other signal that modulates annularly with a similar phase and amplitude, is tricky. So far, several experiments have failed to see dark matter-like signals in the mass and cross section range consistent with DAMA’s observations.
However, dark matter is slippery, and it could always be the case that WIMPs have just the right combination of properties that they might preferentially interact with NaI detectors, or it may be the case that some detail of their interaction mechanism allows them to show up in DAMA’s annular modulation analysis but hides them from other analyses.
The goal of COSINE, then, is to search for the same annular modulation signal using the same kind of detector.
Achieving the sensitivity needed to test the DAMA results requires ultra-pure NaI crystals with a very low energy threshold. This is exactly what they achieved. Conveniently, these conditions also allow them to be sensitive to signals produced by axion-like particles coming from the sun.
What did they find?
Figure 1 in this paper, copied below, illustrates the axion-induced signal COSINE-100 looked for. In particular, as an axion approaches the strong electromagnetic field surrounding a Na or I nucleus, it may couple to a photon and transfer its energy to an atomic electron. This process is called the axio-electric effect, and is very similar to the more familiar photoelectric effect, except much weaker.
When this process happens, the entire energy of the axion is absorbed by an electron, creating a recoiling electron whose energy is the axion’s total energy, minus the atomic binding energy of the electron.
To search for solar-axion induced signals in the detector, they looked at the low energy spectrum observed in each detector. They then compared this signal to that expected from their background model and performed a likelihood-based fit to determine the largest axion-electron coupling constant gae consistent with their observation.
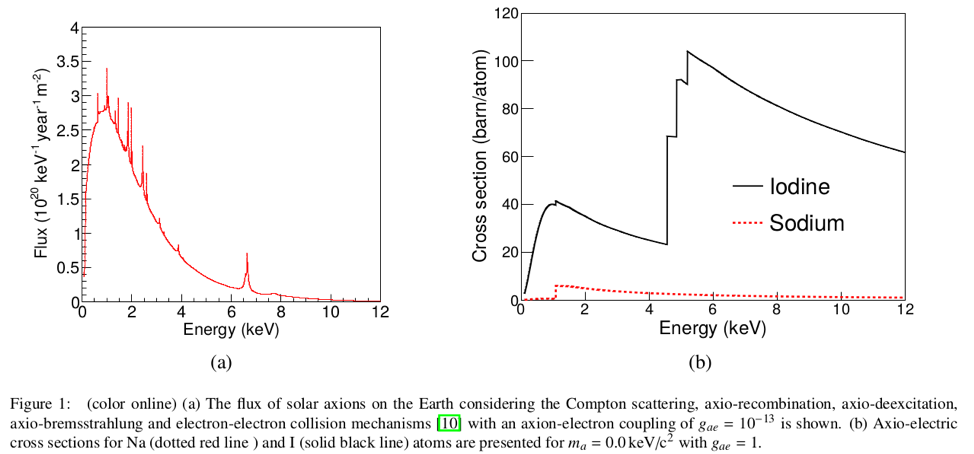
Figure 1 and caption from the paper.
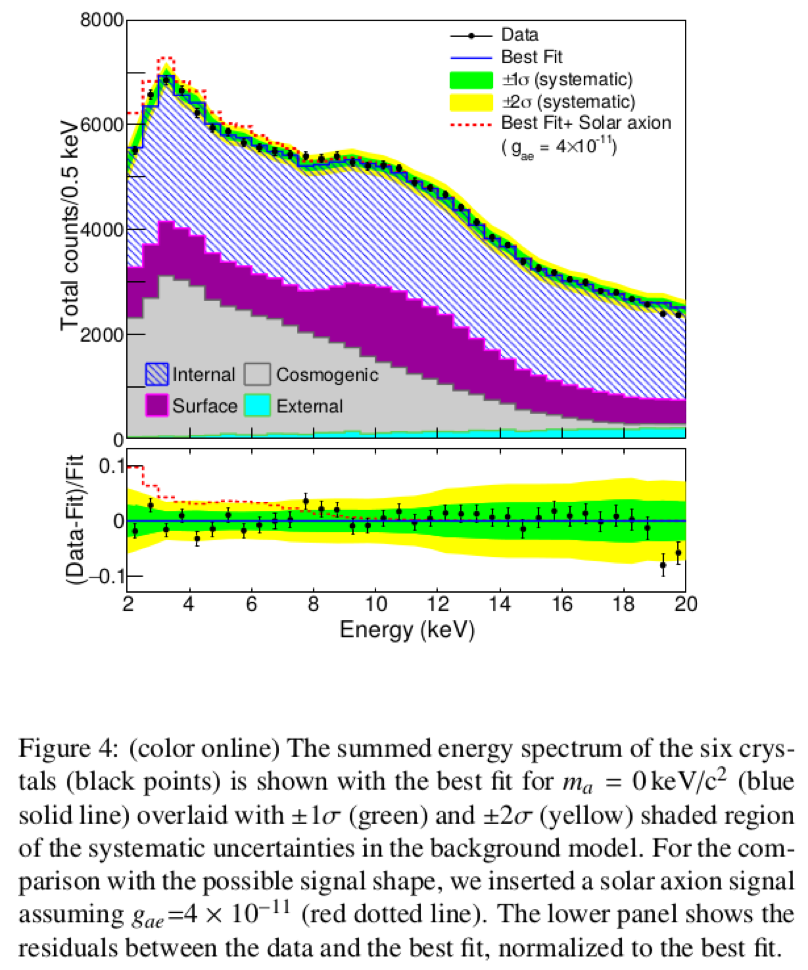
Figure 4 and caption from the paper.
The results from this fit are shown to the left, in the range of 2-20 keV. Based on this fit, they find that gae < 4×10-11 for axions with a mass below 1 keV. For comparison, the spectrum expected for such an axion is shown as well.
As mentioned earlier, there are several different models for axions and axion-like particles that relate their mass and coupling constant. Two of the most popular models for so-called “invisible” QCD axions that can explain dark matter are the DFSZ and KSVZ models (as a tangent, if you consider the now excluded WWPQ model, there seems to be some underlying rule that all axion models must have four letters). The search is performed looking at electron recoil energies above 2 keV. In this analysis, they only consider axion masses below 1 keV/c2, set by the range of validity of the solar axion flux model used. As a result, the search was performed entirely over highly relativistic axions, and simulations indicated very little dependence on the axion mass on the expected signal.
Finally, they put their results into context in the plot on the right. Since their search was more or less independent of the axion mass over the range of masses considered in the analysis, they managed to fit out a flat line of constant coupling constant. Since the DFSZ and KSVF models predict particular relationships between the axion mass and coupling constant, these curves are shown for comparison as well.
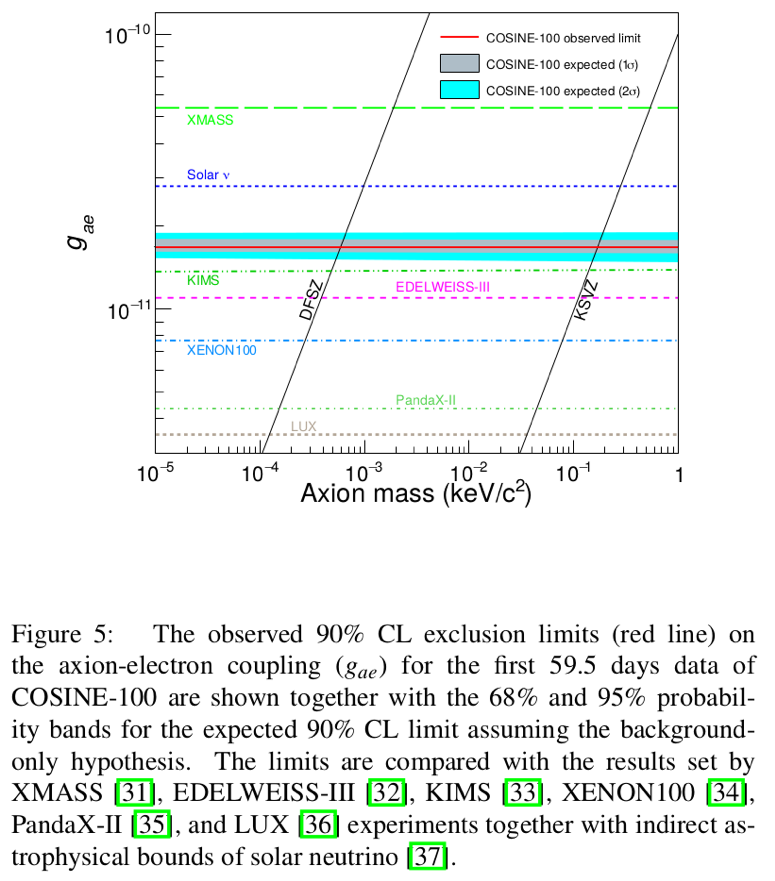
Figure 5 and caption from the paper.
As a result, they can completely exclude QCD axions with mass above 0.59 eV/c2 in the DFSZ model and above 168.1 eV/c2 in the KSVZ model.
Lastly, we note that other experiments have already excluded coupling constants in this region. However, confirmation that COSINE excludes these coupling constants as well, makes the parameter space of allowed models more robust. And clearly the high sensitivity achieved by the collaboration is a sine of good things to come in their other physics analyses.
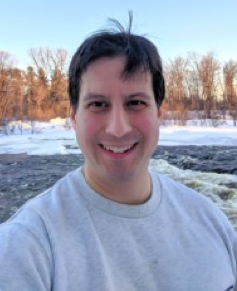
About Shawn Westdale
I am a postdoctoral fellow at Carleton University in Ottawa, working on the DEAP-3600 direct dark matter detection experiment. I completed my PhD at Princeton, working on the DarkSide-50 experiment. And before that, I worked on the MiniCLEAN experiment for my bachelors at MIT. As a hipster physicist, I was into dark matter detectors when they were still underground.