Half-Life 2νECEC Confirmed (in 124Xe)
By Shawn Westerdale, May 22, 2019
Article: “Observation of two-neutrino double electron capture in 124 Xe with XENON1T”
Authors: The XENON Collaboration
Reference: arXiv:1904.11002 [nucl-ex]
This article has been getting a lot of buzz lately in the press, so I figured I’d make a last-minute change to talk about this article, rather than the one I was previously planning to discuss (you’ll have to wait a few weeks to find that one out).
As the title suggests, the XENON1T Collaboration has recently observed the two-neutrino double electron capture (2νECEC) process in 124Xe for the first time ever.
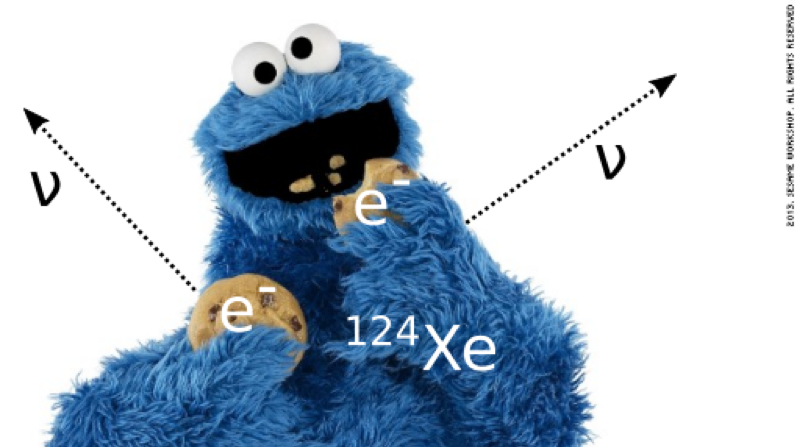
Figure 1: Artistic depiction of the 2νECEC process on 124Xe.
Given all the press coverage, you’ve probably also noticed that this is a pretty exciting result. So what is this process, why is it exciting, and how can it be measured?
What is it?
Electron capture (EC) is a nuclear process by which a proton in the nucleus interacts with an atomic electron to produce a neutron and an electron neutrino.
This can be written as AZ + e– → A(Z-1) + νe,
Where A and Z are the mass number and atomic number of the initial state nucleus, respectively.
Where the initial state electron most likely comes from one of the inner atomic shells. This interaction happens with some Q-value, due to the energy difference of the initial and final state nuclei; this energy is mostly carried away by the neutrino.
However, a sudden change in the charge of the nucleus and the loss of an inner shell electron means the final state atomic electrons are no longer in a ground state configuration. As the electrons reconfigure themselves into the ground state of the daughter nucleus and fall into lower energy orbitals, a cascade of x-rays and Auger electrons are released to carry away the energy difference between the electrons’ initial and final configurations.
If the EC reaction is not energetically favorable (e.g. the rest masses of the initial state nucleus and electron are less than the rest mass of the daughter nucleus), it will not happen. For some (very few) nuclei, EC is not energetically favorable, but simultaneously converting two protons into two neutrons results in a lower energy final state and so double electron capture (ECEC) is possible. This reaction can be written as AZ + 2e– → A(Z-2) + 2νe.
Since this reaction produces two neutrinos, it is referred to as 2νECEC (two-neutrino double electron capture) to distinguish it from 0νECEC (neutrino-less double electron capture). Unlike 0νECEC, 2νECEC is predicted by the standard model; though since it involves the nucleus absorbing two electrons, it is regarded as a second-order weak interaction. There are very few nuclei for which this reaction is expected to be possible, and the fact that this is a second-order process means that these decays are also expected to be extremely rare. Prior to this measurement, 2νECEC had only been observed on two other nuclei, 78Kr and 130Ba. This makes 124Xe the third isotope on which this process has ever been observed.
The isotope 124Xe has a natural abundance just below 0.1%, meaning that a very large volume of xenon is needed to obtain an appreciable mass of 124Xe. Conveniently, the XENON1T detector has about 2 tonnes of sensitive liquid xenon (LXe) and therefore about 2 kg of 124Xe on which to observe this decay.
The reaction can be written as,
124Xe + 2e– → 124Te + 2ν (2.857 MeV)
While the Q-value for this reaction is quite high for these low energy dark matter detectors like XENON1T, detecting these neutrinos is very hard. Instead, the key to detecting this reaction is to detect the 64.3 keV of energy carried away by the x-rays and Auger electrons released by the 124Te electrons relaxing to their ground state after the decay.
Why is it exciting?
The short answer is that it is exciting because it has never been seen before! As mentioned, this is an incredibly rare process, and this is only the third isotope in which it has ever been observed. On top of that, XENON reports a half-life of (1.8±0.5) x 1022 years, more than a trillion times the age of the Universe, making it the longest half-life ever observed in an experiment.
But the interest in these results goes even deeper. First, nuclear decays are one of the more sensitive probes we have to answer questions about nuclear structure and weak interactions. Having a new nucleus to study this interaction on allows for additional constraints on these models.
Furthermore, this gives us another probe to study the elusive and mysterious neutrino. One of the major modern mysteries of the neutrino relates to its basic nature — is it a Majorana particle (equal to its own anti-particle) or is it a Dirac particle (opposite to its own anti-particle)? This is commonly probed by looking for the neutrino-less double β-decay reaction (0νββ) — the opposite of 0νECEC, in which a nucleus simultaneously emits two electrons but no neutrinos. The more common version of this reaction that has been observed before is two neutrino double β-decay (2νββ). According to the Standard Model, under the assumption that neutrinos are Dirac particles, both of these anti-neutrinos must be produced in order to respect lepton number conservation. However, if neutrinos have a Majorana nature, they can cancel out, leaving all of the energy to the more easily detectable electrons. However, as you can imagine, the probability of this happening is expected to be exceptionally tiny, and this process has never been observed. If it were observed, it would be evidence of a Majorana nature to the neutrino. So far, experiments have only succeeded at putting lower limits on the half-life of various candidate isotopes (for example, EXO-200 has measured a lower limit of 1.2 x 1024 years for 0νββ in 136Xe). Translating these upper limits (or potential future half-life measurements) into actual information about the nature of the neutrino requires comparing multiple sets of measurements with different nuclei or different processes.
While the recent observation reported by XENON was of 2νECEC, the fact that they were able to observe it opens the doors for future studies of 0νECEC in their detector. In addition to providing an additional mode through which the Majorana nature of the neutrino can be probed, the finer details of these models can be better understood by comparing searches fo 0νββ to searches for 0νECEC. As such, this observation potentially opens the door for more studies probing the nature of the neutrino.
How can it be measured?
The XENON1T detector is described at length in this paper. The detector itself is located in Laboratori Nazionali del Gran Sasso (LNGS), beneath a mountain in Central Italy. A diagram of the detector is shown below (from arXiv:1708.07051). The detector is primarily intended to be a dark matter detector and runs with 2 tonnes of sensitive LXe with exceptionally low backgrounds.
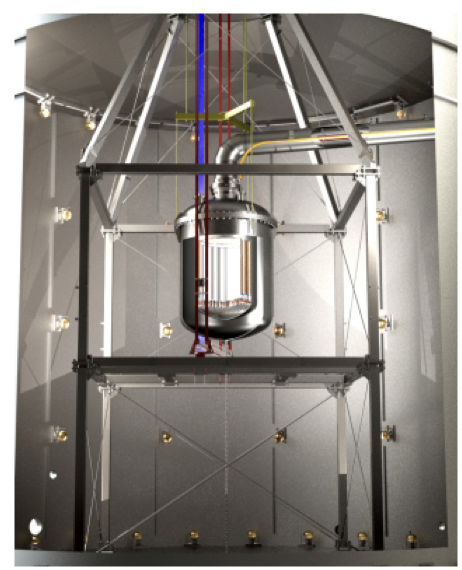
Figure 2: Figure 6 from this paper showing the stainless-steel cryostat containing the LXe time projection chamber (TPC) installed inside a 740 m3 water shield equipped with 84 photomultiplier tubes (PMTs) deployed on the lateral walls. The cryostat is freely suspended on a stainless-steel support frame, which can be converted into a cleanroom around the cryostat. The cryostat is connected to the outside by means of two pipes. The large, vacuum-insulated cryogenic pipe carries all gas/LXe pipes and cables. A small pipe (yellow) is used for the cathode high-voltage. Also shown is the system for calibrating XENON1T with external sources installed in movable collimators attached to belts (blue, red).
An illustration of how the detector works is given in the figure to the right. The basic idea is that the LXe produces a flash of scintillation light (called S1) when something deposits energy into it. At the same time, several electrons are ionized from the xenon atoms and drifted towards the top of the detector in an electric field known as the drift field. When these electrons reach the top of the detector, a stronger field (the extraction field) accelerates the electrons through a layer of gaseous xenon, producing additional light (the S2 signal). By measuring the size of both of these signals, the energy deposited into the detector can be measured. By looking at the time between the S1 and S2 signals, the Z-coordinate can be determined, and by looking at the distribution of light amongst the photomultiplier tubes (PMTs), x- and y-coordinates can be reconstructed as well. For their 2νECEC analysis, a total live time of 177.7 days was used.
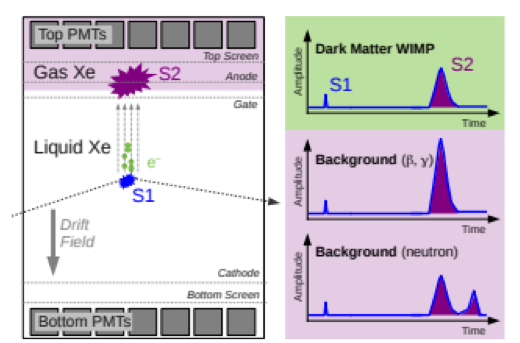
Figure 3: Figure 1 from the paper showing the working principle of a dual phase LXe TPC. The prompt (released immediately after the interaction) scintillation light (S1) generated in the LXe is recorded by PMTs installed above and below the target. The same PMTs also measure the delayed secondary-light signal, S2, which is created by scintillation in the gas phase Xe induced by ionized electrons (e–). A set of TPC electrodes is used to establish the required electric fields. The location of the interaction can be reconstructed in 3-D by using the S2-signal pattern observed by the top PMTs (lateral) and the time difference between S1 and S2 (depth). Background events are rejected by the charge to light (S2/S1) ratio and the scatter multiplicity (number of S2 signals), as indicated on the panels on the right.
The primary external backgrounds to this analysis came from γ-rays from 60Co, 40K, and isotopes in the 238U and 232Th decay chains. These isotopes are naturally present in trace quantities in the detector materials, and the γ-rays they produce may produce low energy scatters in the LXe around the ~60 keV energy region of interest. XENON1T was constructed with strict requirements on the radiopurity of its detector components; these backgrounds are further reduced by identifying events with multiple S2 pulses, which may be a sign of a γ-ray scattering multiple times within a detector — due to the short interaction length of x-rays and Auger electrons, such a signal is not expected from the 2νECEC process. A fiducial volume is also defined to further suppress these backgrounds.
Significant internal backgrounds are also present from the β-decays 214Pb and 222Rn, which may be present in the LXe from radon and its progeny diffusing into the LXe, and from 85Kr, a naturally-present radioactive contaminant. Backgrounds are also present from the 2νββ decay of 136Xe in the detector.
Lastly, rare nuclear reactions in the detector environment may produce neutrons, which can penetrate the detector’s shielding, enter the LXe, and capture on 124Xe to produce 125Xe. 125Xe eventually decays, through 125I, to an excited state of 125Te. This state decays by emitting x-rays and Auger electrons with a total energy of 67.3 keV, very close to the 64.3 keV peak expected for the 2νECEC decay of 124Xe. Fortunately, the 125I has a half-life of 59 days and is removed from the LXe by the purification systems with a timescale just over 1 week. A total of 10 ± 7 events are expected in the energy region of interest from this background source.
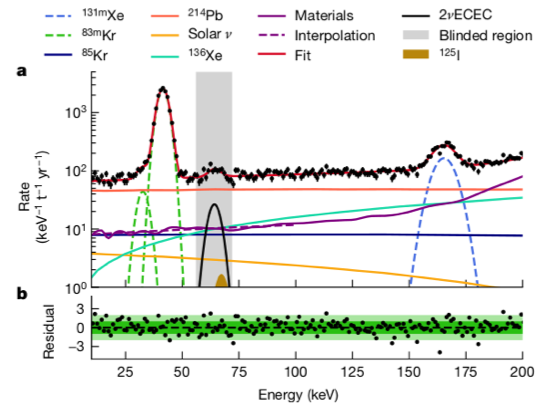
Figure 4: Figure 2 from the paper showing the data (black dots), and the fit to the data (dark red line). The fit uses models for different backgrounds whose individual sources are shown as well. These backgrounds were estimated with the data in the blinded region around the expected 64.3 keV line from the 2νECEC signal. The bottom panel shows the resulting residuals when the data is subtracted from the fit.
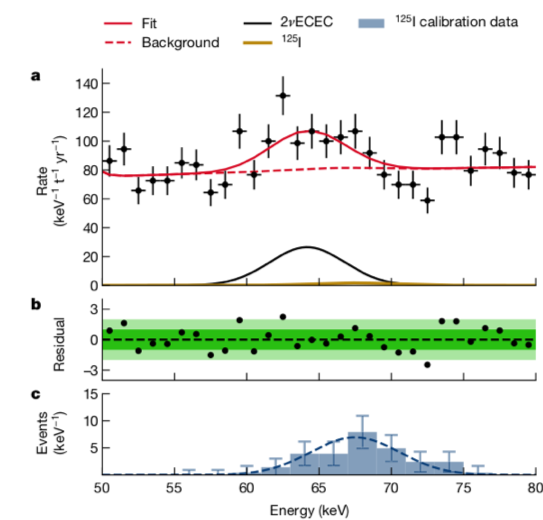
Figure 5: Figure 3 from the paper zooming in on the region of interest in Figure 2. The bottom panel also shows the number of events from the 125I calibration data.
The two plots above show the measured energy spectrum during this data collection period, along with a fit of the background and signal (2νECEC decays at 64.3 keV) models. The plot on the left shows the spectrum in a wide range, while the plot on the right is zoomed into the area around the 2νECEC peak.
A bump is seen at the expected energy with 4.4σ significance, constituting the first ever observation of 2νECEC in 124Xe.
Overall, this paper presents an impressive bit of analysis showing all of the non-dark matter physics that can come out of current dark matter detectors, with their extremely low background rates and high energy resolution. At the end of this paper, the authors point out that future detectors will have the ability to measure this half-life to even higher precision, enabling a better understanding of nuclear structure, and that future analyses may include searches for 0νECEC of 124Xe, as well.
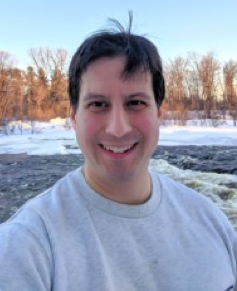
About Shawn Westdale
I am a postdoctoral fellow at Carleton University in Ottawa, working on the DEAP-3600 direct dark matter detection experiment. I completed my PhD at Princeton, working on the DarkSide-50 experiment. And before that, I worked on the MiniCLEAN experiment for my bachelors at MIT. As a hipster physicist, I was into dark matter detectors when they were still underground.