Borexino Collaboration confirms existence of Solar CNO neutrinos
Neutrinos from the Carbon-Nitrogen-Oxygen cycle are a probe for solar energy production, initial state, and metallicity – potentially clearing the experimental path to solving the solar metallicity problem.
McDonald Instant Overview (1 minute read)
- Borexino is a liquid scintillator experiment built for solar neutrino detection, located at the Laboratori Nationali del Gran Sasso in Italy. It is comprised of 278 tonnes of liquid organic scintillator, housed in a 4.25 m radius spherical nylon vessel, surrounded by ~600 tonnes of ultra-pure organic buffer liquid to shield the residual radioactivity of 2212 Photomultiplier tubes (PMT). Solar neutrino signals emerge from the events occurring within the innermost, most radio-pure fiducial volume (FV) of approximately 100 tonnes. This is not a physical boundary, and the fiducial volume is optimized for each search. Borexino started taking data in May 2007 and is the most radio-pure neutrino detector in the world.
- The CNO cycle is the primary mechanism for solar hydrogen to helium fusion in the universe. Even though it only accounts for 1% of the energy output from the Sun, direction detection provides information about the metallicity and initial state of the Sun. Additionally, knowledge about the solar CNO cycle may assist in solving the solar metallicity problem.
- Borexino confirmed their detection of CNO neutrinos on June 2020, with a publication in Nature appearing on November 25 2020. The reported interaction rate for CNO solar neutrinos is \( 7.2_{-1.7}^{+3.0}\) counts per day per 100 tonnes of target at the 68% confidence level. This corresponds to a neutrino flux on Earth of \( 7.0_{-2.0}^{+3.0} \times 10^8 cm^{-2}s^{-1} \), with the absence of a CNO neutrino signal disfavoured at \( 5\sigma\).
What is Borexino?
The Boron Solar Neutrino Experiment (Borexino) is located at Laboratori Nazionali del Gran Sasso, near L’Aquila, Italy. The experiment is supported by researchers from Italy, the United States, Germany, France, Poland, and Russia. It utilizes elastic scattering on the electrons of the liquid scintillator to detect low energy solar neutrinos, and is also part of the Supernovae Early Warning System (SNEWS). It began taking data in May 2007, and has undergone several upgrades to improve radio-purity, temperature control, and background characterization.
The detector has an active core of 278 tonnes of pseudocumene (PC) and 2,5-Diphenyloxazole (PPO). Pseudocumene is an organic, aromatic hydrocarbon that is isolated during petroleum distillation and often used as a sterilizing agent or in detergent, dyes, perfumes, and resin. It is also known as 1,2,4-Trimethylbenzene, C6H3(CH3)3. PPO, or 2,5-Diphenyloxazole, C15H11NO, is an organic scintillator and wavelength shifter whose output spectrum peaks at 385 nm and is added to PC to convert the scintillation light to a longer wavelength to which the mix is transparent.
18 m wide and 16.9m tall, Borexino is constructed of two main concentric spheres inside a large water tank. The inner sphere is made of transparent nylon and holds the liquid scintillator, with a radius of 4.25 m. The outer sphere is stainless steel with a radius of 6.85 m. The spherical shell between the liquid scintillator nylon boundary and the stainless steel boundary is filled with 1000 tonnes of high-purity pseudocumene. 2212 photomultiplier tubes (PMTs) are mounted on the inner surface of the stainless steel sphere, 1800 of which are specifically observing the scintillator volume. Outside the stainless steel sphere is a 2400 tonne pure water buffer.
As with all neutrino detectors using PMTs, the detector’s energy and spatial resolution for events is deteriorating with time due to the loss of PMTs. As of June 2020, the average number of active channels was 1238 with energy resolution \(\sigma_E/E \approx 6\%\) and spatial resolution \(\sigma_{x,y,z} \approx 11 cm\) for a 1 MeV event in the centre of the detector.
Borexino has set limits on parameters for sterile neutrinos, electric charge conservation, and solar activity stability within the past decade. It has detected geoneutrinos and solar neutrinos from 7Be, 8B, p-p, p-e-p, and most recently, CNO processes. To have detected CNO neutrinos amidst numerous neutrino background sources, Borexino needed to focus on extreme radio-purity and fine-tuned background and signal characterization.
 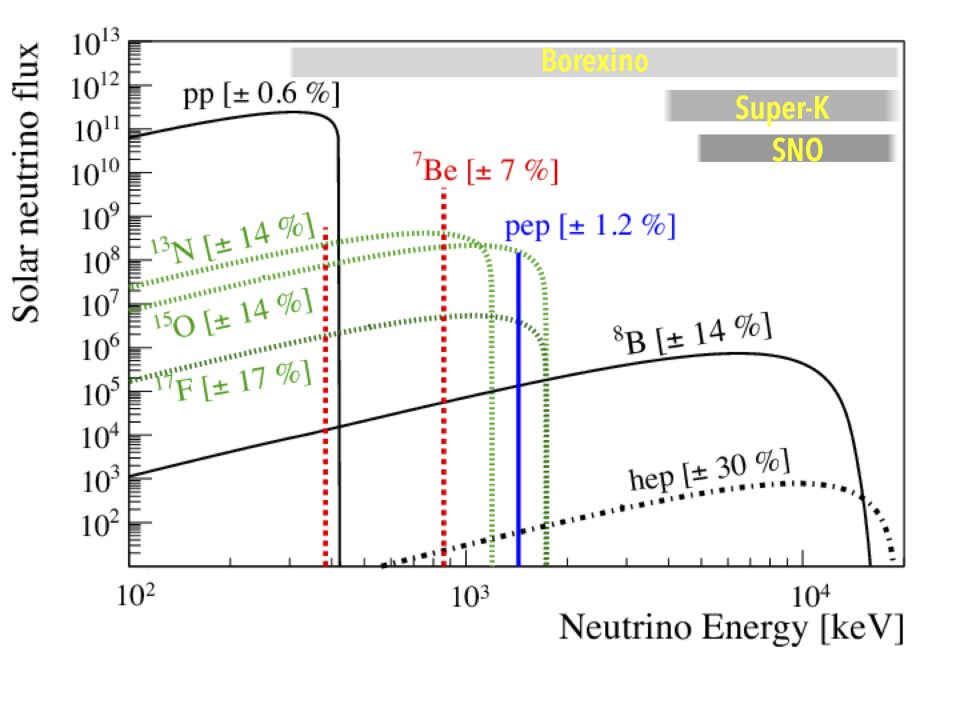
The gray bands compare the regions where the three solar neutrino telescopes, that are able to measure the energy of the events, are sensitive. Note that the predictions of solar models are in logarithmic scale: Super-Kamiokande and SNO can observe about 0.02% of the total, while Borexino may observe each type of predicted neutrino. (Francesco Vissani, https://creativecommons.org/licenses/by-sa/4.0)
Why is Borexino considered the most radio-pure detector in the world?
Borexino has extremely high radiopurity due to its purification techniques, cleaning protocols, and selection of materials. Based on the graded shield technique, there are lower amounts of intrinsic radioactivity in successive layers of the detector. So much so, that the 278 tonnes of liquid scintillator in the core is further segmented into 100 tonnes of FV surrounded by 178 tonnes of low-background scintillator buffer.
In line with the graded shield technique, the pure pseudocumene layer is further split by a nylon barrier at radii 5.50 m and 6.85 m, creating two layers of pure pseudocumene buffer fluid. This double buffer shields the active scintillator core from 𝞬 radiation emitted by potential contaminants in the PMTs and outer stainless steel sphere. The outermost nylon vessel provides a barrier against Radon emanated from the PMTs that could otherwise migrate to the vicinity of the scintillator.
The main materials used in the construction of Borexino are nylon, plastic supports for the nylon vessels, copper, steel, glass for the PMTs, and aluminum light concentrators. The construction, transport, and assembly of the detector components followed strict steps to minimize contamination. The scintillator in particular has undergone two rounds of purification; once in 2007 with low-argon-krypton nitrogen stripping and distillation during detector filling, and in 2010-2011 with several cycles of ultra-pure water extraction and further stripping.
The location of Borexino is also a major component of its impressive radiopurity. The Gran Sasso d’Italia mountain range boasts the second tallest peak in Italy, after the Alps, at 2912 m above sea level. Most of the Gran Sasso mountain range belongs to parks, conservation areas, and ski resorts. The Laboratori Nationali del Gran Sasso is located in Assergi, with Borexino buried under 1400 m of rock or 3500 m of water equivalent. This depth of rock shields the cosmic rays that hit the surface of the Earth and suppresses their muon component by approximately one million-fold.
  What are Borexino's characterized backgrounds?
Due to the location, materials, cleaning procedures, purification techniques, and design, Borexino is the most radiopure neutrino detector in the world. Backgrounds from the construction materials and external shieldings are therefore negligible, and background characterization can focus on intrinsic contamination of the scintillator itself. The only significant external radiation affecting Borexino is that from penetrating muons.
In Phase II of the experiment (2012-2016), backgrounds from 85Kr, 210Br, 210Po, 40K, and cosmogenic 11C were well characterized, along with 𝞬 decay of 40K, 214Bi, and 208Tl external to the scintillator. Pulse-shape discrimination methods were used to time profile light and distinguish between 𝞪, 𝞫+, and 𝞫– particles. This was essential for 210Po 𝞪 decays and constraining the 210Bi background.
Muon tagging (external)
The water tank exterior of Borexino serves two purposes; to shield the detector from external radiation and to tag residual cosmic muons. The water tank has 200 outward pointing PMTs in addition to the 2200 PMTs mounted on the interior of the stainless steel sphere.
The PMTs mounted on the interior of the stainless steel sphere are comprised of 1800 with light cones, and 400 without. The 1800 with light cones are configured such that they only observe light from the nylon sphere region that contains the FV, while the 400 without light cones are sensitive to the total volume of the stainless steel sphere. This enables the detection of muons that only cross the pure pseudocumene buffer layers.
Scintillator background (internal)
The 210Bi and p-e-p neutrino backgrounds are the most significant in regards to the detection of CNO neutrinos in Borexino. The neutrino contribution from the p-e-p process may be constrained to the 1.4% level from solar luminosity, however the 210Bi background is significantly more nuanced.
210Bi can be constrained by measuring 210Po due to the process
\( ^{210}\rm Pb \; \rightarrow \; ^{210}Bi \; \rightarrow \; ^{210}Po \; \rightarrow \; ^{206}Pb\).
The half-life of 210Bi here is 5 days and the emitted 𝞫- particle energy is too low to be detected in Borexino. The half-life of 210Po is 134.8 days and the emitted 𝞪 particle can be detected, therefore making it an ideal tracer for 210Bi. However, this is only valid if the above process is in secular equilibrium.
Two sources of 210Po were distinguished in the detector: that in the scintillator, and that from the vessel. The vessel sources are deposits on the interior surface of the scintillator vessel that may become dislodged, diffused, or displaced by convective currents. No evidence of leaching outwards from the scintillator volume was found, and diffusion was found to be a negligible effect. Convective currents from temperature gradients were found to be significant for the movement of 210Po in the FV in Phase II.
Fluctuating rates of 210Po were seen in the FV due to convection currents until 2016 when temperature control and thermal insulation mechanisms were installed. Borexino was installed on a cold floor, or rather directly onto the rock of the mountain, which acts as an infinite thermal sink. The detector was additionally thermally insulated against the air temperature in the lab with the installation of insulation around the water tank with a thermal conductivity of 0.03 Wm-1K-1. An active control system further compensates for any change in detector temperature with a precision of 0.07℃. With these considerations, a stable positive temperature gradient was achieved in the scintillator ranging from 7.5℃ at the rock base to 15.8℃ at the top with seasonal modulations of 0.3℃ every 6 months.
Minimizing the convection currents led to the “Low Polonium Field” (LPoF) in the upper hemisphere of the scintillator. The upper limit of 210Bi is determined from the minimum 210Po rate in the LPoF via
\(\rm R(^{210}\rm{Po}_{\rm min}) = R(^{210}\rm{Bi}) + R(^{210}\rm{Po}_{\rm V})\),
where 210PoV is from the vessel. It was found that the 210Pomin rate was not a statistical fluctuation, and statistical uncertainty for the spatial non-uniformity of 210Bi could be constrained to 0.78 counts per day per 100 tonnes. This was consistent with 2D and 3D numerical simulations of the scintillator volume, leading to an overall upper limit of \( \rm R(^{210}Bi) \leq 11.5 \pm 1.3 \) counts per day per 100 tonnes.
 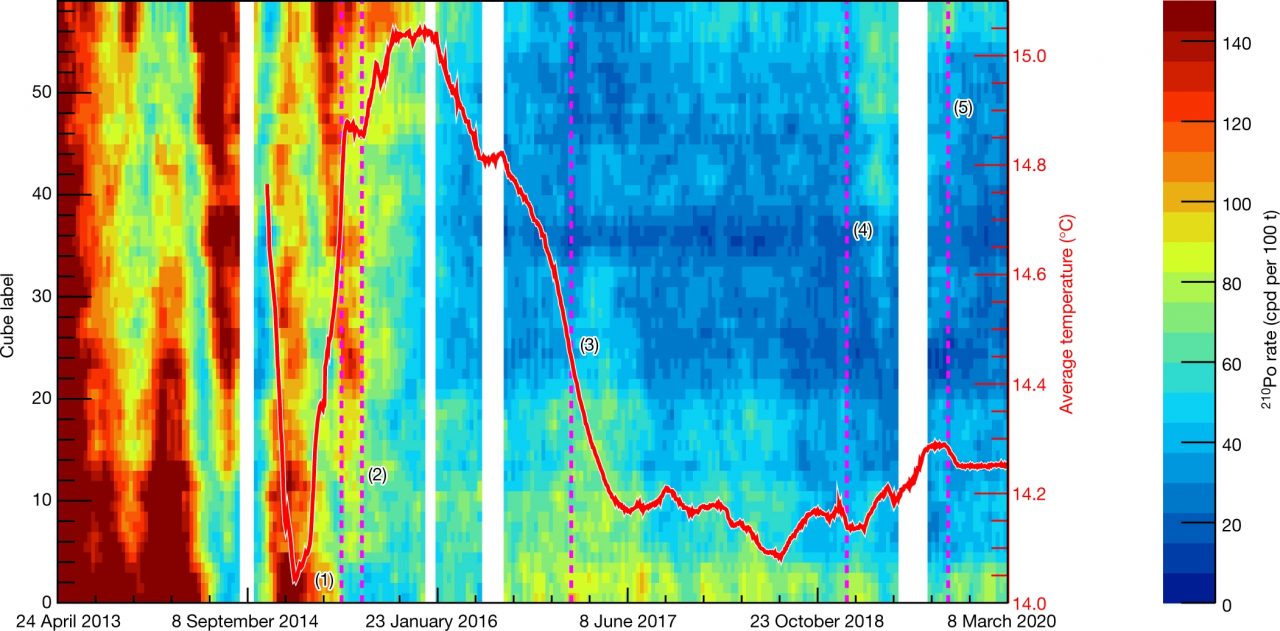
(Figure 3 in June 2020 publication) 210Po rate in Borexino in cpd per 100 tonnes (rainbow color scale) as a function of time in small cubes of about 3 tonnes each ordered from the bottom, “0”, to the top, “58”, along the vertical direction (Latest update: May 2020). All cubes are selected inside a sphere of radius r = 3 m. The red curve with its red scale on the right represents the average temperature in the innermost region surrounding the nylon vessel. The dashed vertical lines indicate the most important milestones of the temperature stabilization program: 1. Beginning of the “Insulation Program”; 2. Turning off of the water recirculation system in the Water Tank; 3. First operation of the active temperature control system; 4. Change of the active control set point; 5. Installation and commissioning of the Hall C temperature control system. The white vertical bands represent different DAQ interruptions due to technical issues.
Rigour of Data Analysis
The data analyzed for evidence of CNO neutrinos is from Phase-III of the Borexino experiment (June 2016 – February 2020), which constitutes 1072 days of live detector time. The neutrino counts were filtered based on residual radioactive impurities, cosmic muons, cosmogenic isotopes, electronics noise, and external 𝞬 background. The considered scattered electron energy band ranged from 0 to 1.517 MeV, in which the region of interest was 0.780 – 0.885 MeV. The volume the analysis focused on was a 71.3 tonne subset of the FV. The rates for the 3 neutrino flavour components from the CNO processes were combined by fixing the ratio between them using the standard solar model.
To extract the CNO neutrino count from other sources, a multivariate analysis was performed in the narrowed energy band 0.32-2.64 MeV. Free parameters included CNO, 85Kr, 11C, 40K, 208Ti, 214Bi, and 7Be neutrinos. P-e-p neutrinos were constrained to 2.74±0.04 counts per day per 100 tonnes, and 210Bi unconstrained within [0, 11.5±1.3] counts per day per 100 tonnes. The reference spectral and background species were obtained with Borexino-specific package based on GEANT-4.
Considering all sources of error, the significance of the CNO observation is \(5.1\sigma\). Possible sources of systematic error were investigated and found to be negligible compared with the CNO neutrino statistical uncertainty. Accounting for uncertainty, the 68% confidence interval of CNO neutrinos is 5.5 – 10.2 counts per day per 100 tonnes. This is consistent with both low metallicity and high metallicity standard solar model predictions.
Assuming Mikheyev-Smirnov-Wolfenstien conversion, neutrino oscillation parameters, and the density of electrons to be \(3.307 \pm 0.015 \times 10^{31} \)per 100 tonnes, the 68% confidence interval of CNO neutrinos may be translated to a CNO neutrino flux on Earth of \(7_{-2}^{+3} \times 10^8 cm^{-2}s^{-1}\).
 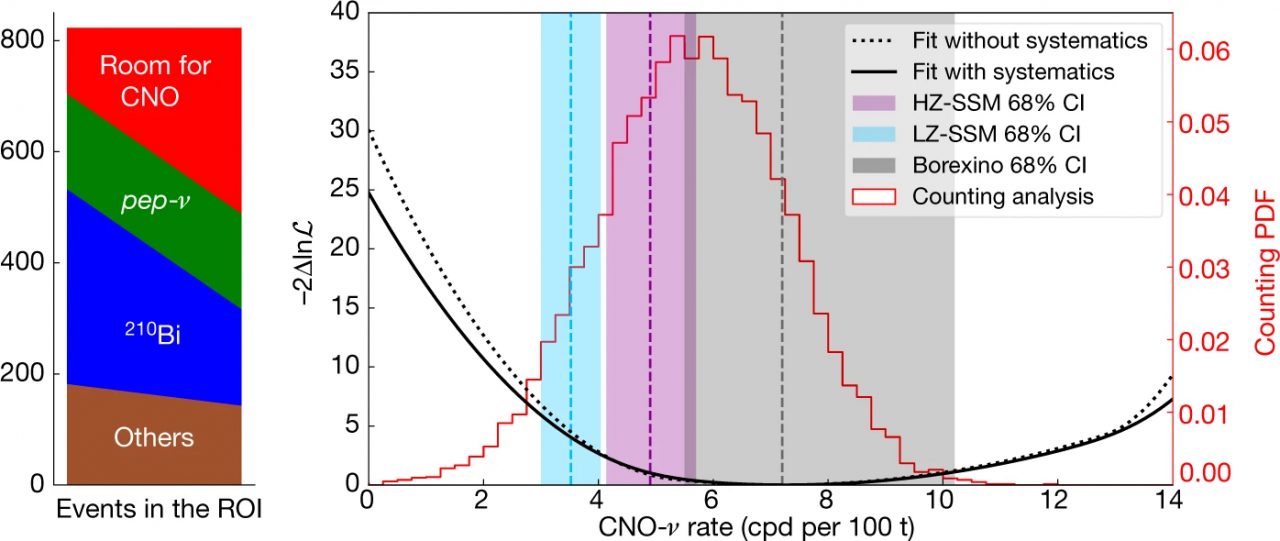
(Figure 5 from June 2020 publication) Left: Counting analysis. The vertical axis is the number of events allowed by the data for CNO-𝝂 and backgrounds in ROI; on the left, the CNO signal is minimum and backgrounds are maximum, while on the right, CNO is maximum and backgrounds are minimum. It is clear from this figure that CNO cannot be zero. Right: CNO-𝝂 rate negative log-likelihood profile directly from the multivariate fit (dashed black line) and after folding in the systematic uncertainties (black solid line). Histogram in red: CNO-𝝂 rate obtained from the counting analysis. Finally, the blue, violet, and grey vertical bands show 68% confidence intervals (C.I.) for the SSM-LZ and SSM-HZ predictions and the Borexino result (corresponding to black solid-line log-likelihood profile), respectively.
What does this mean for APP research?
Detecting and characterizing the full range of solar neutrinos has been a goal for neutrino detectors for decades. Characterizations and analysis of p-p and p-e-p neutrinos is considered well understood, but CNO neutrinos are considerably lower energy.
The CNO cycle is the primary mechanism for hydrogen to helium fusion in the universe due to the abundance of high mass, rapidly evolving stars. It is estimated that in a smaller star such as our Sun, the CNO cycle contributes on the order of 1% to the total solar energy output. There is significant uncertainty around this estimate for our Sun specifically due to ill-constrained metallicity. Metals (anything larger than helium) directly catalyze the CNO cycle, and therefore understanding the role of the CNO cycle in the Sun is tantamount to knowing the metallicity of the Sun.
In addition to information about energy production, metals affect the plasma opacity of the Sun. This in turn affects the core temperature, evolution, and density profile. Due to their far-reaching impacts on the solar state, metals may also be used as a tracer for the initial formation state of the Sun. This would give insights into the history and formation of our solar system. As of now, the metallicity of the Sun is unknown and observations have given rise to the solar metallicity problem; a high metallicity is needed for the Sun to produce the features seen, but the detected surface metallicity is much lower than predicted.
Now that CNO neutrinos have been confirmed and an estimate placed on their flux and count, constraining this measurement and obtaining better estimates is a clear next step. A more precise CNO neutrino flux measurement would qualify as experimental confirmation of the overall solar picture, and lead to a determination of the metallicity of our Sun’s core.
Borexino is not the only neutrino detector looking for CNO and other low-energy neutrinos. SNO+ is also participating in the search. Additional goal posts in this energy range are determining the properties of the Mikheyev-Smirnov-Wolfenstien effect and neutrino oscillation – a continuation of the original SNO legacy and work of Arthur B. McDonald.