Education Hub
Neutrino Physics
In 2015, Arthur B. McDonald co-won the Nobel prize in physics for solving the Solar Neutrino problem. In order to understand how exciting this is, we first need to explore neutrinos themselves, and what they are.
Matter is made up of many tiny pieces called atoms, and believe it or not, atoms are actually mostly empty space! They consist of protons and neutrons, which stick together and make up the middle of the atom called the nucleus. Electrons are particles that orbit the nucleus. Check out this interactive activity where you can build your own atoms to learn more!
It would be quite simple, yet much less interesting, if that’s where this conversation ended. In reality, however, protons, neutrons, and electrons are not the smallest particles that exist! All of the particles that we know of so far are described by the standard model of particle physics. Take a look at the diagram below. In it, you’ll see that there are no protons or neutrons. Instead, you’ll see quarks, a type of “sub”-atomic particle. It turns out protons and neutrons are made of different numbers of the lightest quarks, the up and down quark.
 Standard Model of Particle Physics.
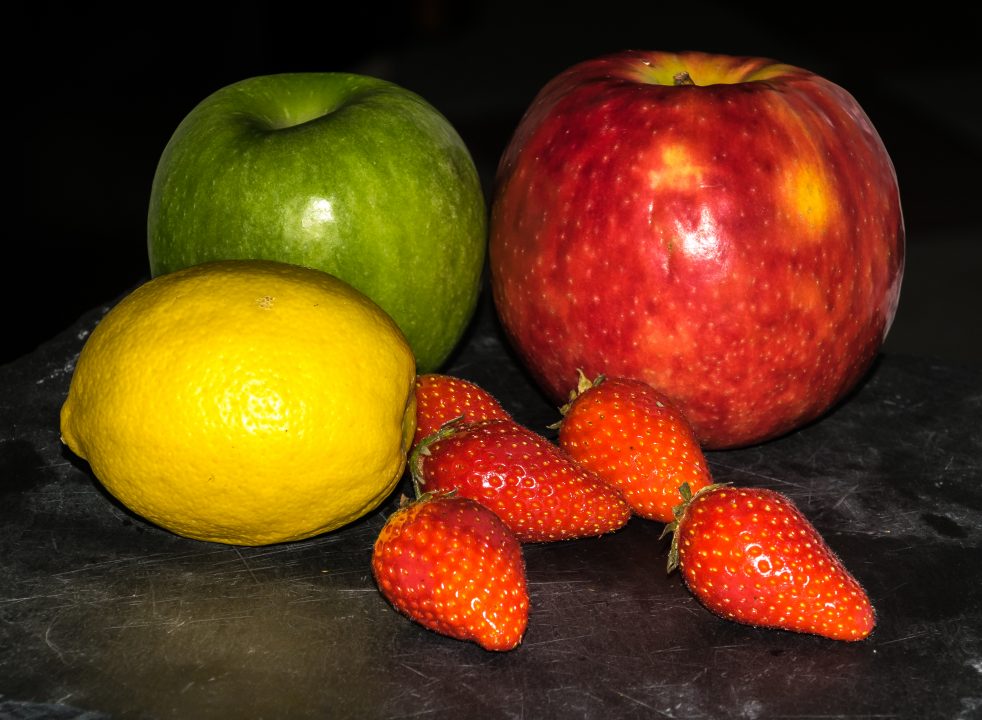
Now let’s talk about the neutrinos. Neutrinos are really tiny subatomic particles that are very difficult to detect, because they don’t interact often with matter. In fact, about 100 trillion neutrinos pass through your body every second! They come in three types which scientists call flavours; electron, tau, and muon. Imagine them as apple-, lemon-, and strawberry-flavoured neutrinos!
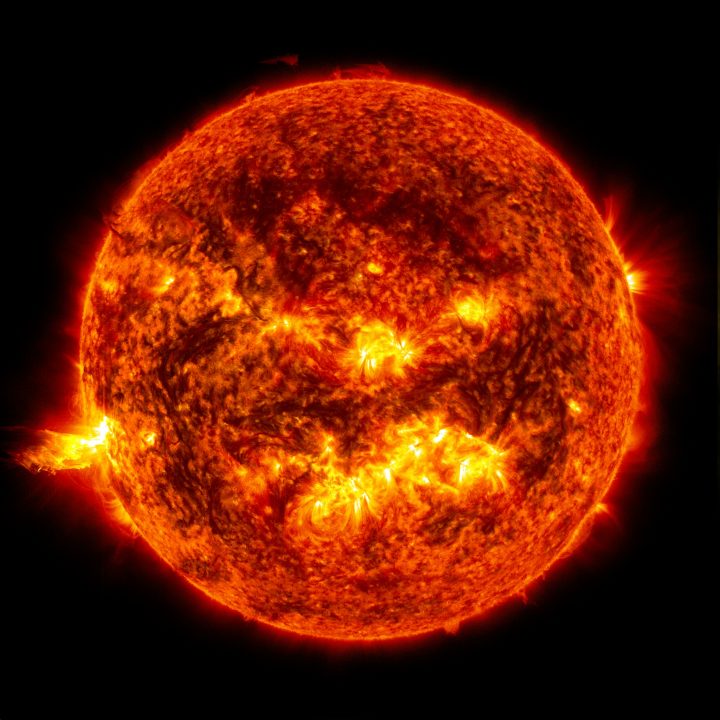
The whole idea of the solar neutrino problem is that scientists predicted that there should be more apple-flavoured neutrinos created by the sun than they were actually detecting on earth by specially-designed apple flavoured neutrino detectors. If the rest of the neutrinos weren’t able to be found, this could be evidence that we don’t understand the sun, or worse, that the sun may be burning out!
Then, in 2002, results from the SNO experiment showed that neutrinos actually change flavour, which means that on their way from the sun they can change from being one type of particle to another. To understand this, let’s imagine that you throw three apples out of your window to a friend. Halfway through the air, one turns into a strawberry, and another turns into a lemon! Then when you ask your friend how many apples they caught, they say one. This would puzzle you, because you thought they’d catch three apples! This is what happens to neutrinos when they travel from the Sun to the Earth. The discovery that neutrinos can change flavour meant that neutrinos have a small amount of mass, and this explains where all the missing neutrinos are, ultimately solving the solar neutrino problem! The neutrinos are still emitted as expected, we just weren’t detecting the ones that changed their flavour!
Learn more about the neutrino experiments McDonald Institute researchers are working on.
 SNO+
SNO+ is a neutrino detector re-using the vessel and underground space from the original SNO experiment. Rather than the heavy water used in SNO, SNO+ uses liquid scintillator which gives off light at the slightest interaction. This makes SNO+ far more sensitive to neutrino interactions than SNO. One of the goals of SNO+ is to be the first experiment to detect neutrinoless double beta decay.
IceCube
IceCube is a neutrino detector that is embedded in the ice in Antarctica. It consists of sensors buried up to 2.5 km below the surface in a cubic kilometre of ice. The ice acts as shielding to filter out unwanted particles, so that most of those that reach the area around the sensors are neutrinos. When a neutrino interacts in the ice, it creates a shower of secondary particles and light that are picked up by the sensors. Using information about the particles and light created, scientists can learn more about the neutrinos.
nEXO
The nEXO experiment will use five tonnes of enriched xenon to learn more about neutrinos. The main goal of the experiment will be to determine if the neutrino is its own antiparticle. Additionally, nEXO will try to determine the mass of neutrinos and attempt to observe neutrinoless double beta decay. NEXO is currently in design, and is estimated to be installed and taking data at SNOLAB in 2025.
HALO
The Helium and Lead Observatory (HALO) is a dedicated neutrino detector that uses 79 tonnes of lead and helium-3 neutron detectors. When a supernova explodes, it releases a burst of neutrinos. These neutrinos reach Earth before the supernova light does. HALO is part of the worldwide Supernova Early Warning System (SNEWS) that alerts astronomers around the globe when a supernova neutrino burst is detected, allowing them to point their telescopes at the supernova in time to observe its light.
P-ONE
The Pacific Ocean Neutrino Explorer (P-ONE) is a new initiative which aims to redevelop ocean-based neutrino telescopes. P-One will harness one of the largest comprehensive ocean observing infrastructures in the world, Ocean Networks Canada (ONC). It is proposed to be a segmented system with several cubic-kilometres of instrument volume optimized for high-energy astrophysical neutrinos, over 50 TeV.